ENVIRONMENT
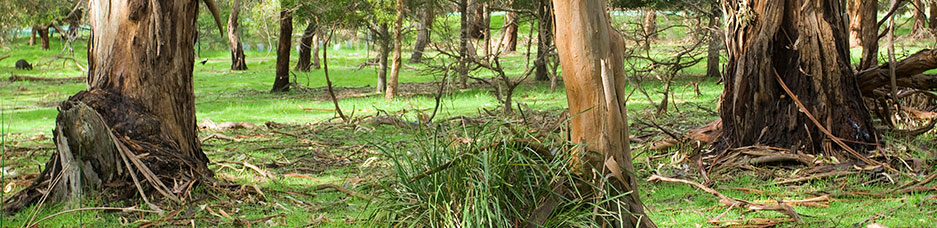
Natural Environment Research Context
Referred to as the New Digital Age (Schmidt and Cohen 2013), or era of Big Data (e.g. Boyd and Crawford 2012; Mayer-Schonberger and Cukier 2013) the present time period provides unprecedented opportunities for a deeper understanding and appreciation of our global environments. The volume of digital data on natural environments has grown exponentially, especially in the physical (e.g. Lynch 2008; Bell et al. 2009) and environmental sciences (e.g. Porter et al. 2012) where much of it is collected by sensors. In addition, data availability has vastly improved as governments in many countries adopt open data policies (Zuiderwijk and Janssen 2014).
Ubiquitous internet technologies provide access to all this data, particularly when that information is delivered on demand via high speed broadband to mobile tablet devices. These technologies have created a society that is spatially enabled and aware. Big business and citizens increasingly expect to be able to access past and current information about any location of interest, to find answers to their spatial queries. Deeper appreciation and understanding comes from the sheer volume of information that can be amassed about any particular place in the landscape, and how that information can be dynamically synthesised to provide the most definitive answer to the user's question.
Hence the use of spatial digital technologies for a more holistic understanding of river catchments, landscapes, biodiversity and environmental sustainability are rapidly emerging areas of eResearch. Recent examples in the international scientific literature include: the UK's Environmental Virtual Observatory project (EVO) which aims to make environmental data more visible and accessible for catchment management (Gurney et al. 2011) and includes visualisation tools for communicating flood risk (e.g. Wilkinson et al. 2013); the web delivery of interoperable water data with Open Geospatial Consortium (OGC) standards (e.g. Boston et al. 2015); landscape visualisations that integrate GIS with augmented reality (e.g. Ghadirian and Bishop 2008); and 3D landscape visualisations for participatory landscape planning (e.g. Wissen et al. 2008; Salter et al. 2009). Experimental tools that use sensors for weed management (e.g Thorp and Tian 2004; Berge et al. 2012), or soil moisture (e.g. Phillips et al. 2014); or monitoring fauna (e.g. Di Cerbo and Biancardi 2013), have also been developed. Similarly, experiments with novel deployment of sensors, such as using unmanned vehicles to improve environmental data collection (e.g. de Sousa and Andrade Goncalves 2011); portable laser scanners for environmental monitoring (e.g. Zlot et al. 2014) and 3D, 4D and augmented reality visualisations, usually at iconic heritage sites (e.g. Guhathakurta et al. 2009; Norris et al. 2014; Petty et al. 2014), have also been published. And a plethora of websites and mobile applications (Apps) are now available to assist the ecologist, farmer, pedologist and hydrologist in on-ground decision making.
With the global shift to smaller governments, an increasing volume of data and most of the decision tools (such as Apps) are now generated in the private sector, particularly the service industries in the environmental, water, energy and agricultural sectors.
The Value of Citizen Contributed Data
Globally, the volume of community-based monitoring data or citizen science data has also grown exponentially since the technologies have empowered the broader community with the means to easily record spatial data (e.g. Conrad and Hilchey 2011; Sui et al. 2013). In response to this, the published research into various aspects of citizen science programs has also proliferated.
The majority of published research in the international scientific literature documents examples of community-contributed biological and ecological data (e.g. Cohn 2008; Couvet et al. 2008; Silvertown 2009), especially the importance of citizen science to ornithological research (e.g. Bonney et al. 2009; Tulloch et al. 2013). Some examples of community based monitoring are impressive, such as the contributions of over 1,000 volunteers to assess the presence of invasive crab species along a 752 km coastal transect across seven states on the east coast of America (Delaney et al. 2008). Community based water quality monitoring in waterways is also universal (e.g. Overdevest et al. 2004; Conrad and Hilchey 2011), such as the Creek Watch program in North America (e.g. Kim et al. 2011).
Apart from contributing monitoring data, the value of citizen science and community-based monitoring to societal education and improved understanding of our planet is also well documented (e.g. Bonney et al. 2014). Citizen science has been contributing data for more than a century and the value from these longitudinal environmental monitoring data sets is only just being realised (Miller-Rushing et al. 2012). Within the science community, questions arise about the integrity of the data collected by citizen scientists, which has also been the subject of much research (e.g. Sheppard and Terveen 2011; Foody 2013). In almost all cases that were reviewed, the consensus is that with appropriate protocols, supervision and training, the data collected by volunteers are equal to that collected by experts (Cohn 2008; Bonney et al. 2014; Danielsen et al. 2014; Castilla et al. 2015; Little et al. 2015; Rossiter et al. 2015).
The motives and categorisation of citizen scientists is another topic of global research. Public participation can be categorised as contributory, collaborative or co-created, depending on whether the program was designed by scientists or collaboratively, and the roles played by the community monitors (Bonney et al. 2014). Citizen scientist typologies have been extended to include the tasks performed, such as observation, measurement, data entry, crowd computing and analysis (Wiggins and Crowston 2012; Haklay 2013), resulting in a number of suggested frameworks for citizen science engagement (e.g. Pandya 2012; Haklay 2013). Motivations for participation vary (Lawrence 2006; Conrad and Hilchey 2011; Rotman et al. 2012) with personal interests and external factors, such as attribution and acknowledgement, important. Also included is concern about anthropogenic impacts on the environment and concern about the effectiveness of government monitoring in the face of funding cutbacks (e.g. Pollock and Whitelaw 2005; Conrad and Daoust 2008).
More recent research publications document studies into the use of spatial digital technologies in citizen science and community-based monitoring. The use of emerging technologies has been analysed (Newman et al. 2012), especially mobile technologies to collect and record spatial data (Graham et al. 2011; Connors et al. 2012; Lowry and Fienen 2013). The value of social networking tools has been compared to traditional media channels (Robson et al. 2013), and concluded that they had some success in increasing and maintaining user participation. Numerous Web geographic information system (GIS) mapping portals aimed at crowdsourcing data have been documented (e.g. Newman et al. 2010; Fienen and Lowry 2012; Werts et al. 2012) and the user-friendliness of these web mapping systems is recognised as a critical encouragement to participate. Research by Newman et al. (2010) in particular resulted in a list of very useful design guidelines. In their research they concluded that ease of useability and user feedback is essential, along with features that target user needs, support the roles of the program and control data quality. Flexible architecture of the web based mapping systems allows them to support multiple citizen science programs without the need to custom design a system for each specific use (Newman et al. 2011).
Interoperability and Dynamic Visualisation
Paradoxically, despite this unprecedented access to record volumes of data, limitations remain on how to use these data to best develop management policy and further the public understanding of environmental science (e.g. Loch et al. 2014). These limitations largely result from the distributed custodianship of environmental data, each with disparate databases and formats resulting in considerable challenges in data discovery, access and harmonisation for the end-user. For example, although there is an impressive volume of Victorian environmental data, information and knowledge available (in both the public and private sectors), few (if any) users have the capacity or desire to handle the data access issues. Combining the diverse datasets into a single web-based data portal has been out of scope for Victorian government departments and beyond the capacity of individual users. This is likely to apply to other State governments.
A solution to this challenge is provided through digital data interoperability, which provides the capacity to transfer and use data held in disparate databases in a uniform and efficient manner across multiple organisations and information technology systems. The systems rely on Spatial Data Infrastructure (SDI) that uses communication protocols to achieve technical interoperability, common data models to achieve schematic interoperability and the use of controlled vocabularies to achieve semantic interoperability (Brodaric and Gahegan 2006). The key aspect of these interoperable systems is that the source data resides with the custodians and is accessed on demand through the internet, ensuring its currency and validity.
Globally, there are government initiated examples such as the European Commission's INSPIRE specifications (INSPIRE 2013a; 2013b); the United States water monitoring networks (ACWI 2013; Blodgett et al. 2015; Brodaric et al. 2015); the French ‘Water Information System’ (BDLISA 2013; BDLISA 2014; ADES 2015), the New Zealand SMART system (Klug and Kmoch 2014; Kmoch et al. 2015); Canadian ‘Groundwater Information Network’ (Boisvert and Brodaric 2012; Brodaric et al. 2015); and the Australian 'National Groundwater Information System' (BOM 2015; Carrara et al. 2015). These portals each provide current environmental data, dynamically federated from authoritative and trusted sources and in some cases, include tools that allow spatial data to be visualised in 3D.
This approach has also been adopted at CeRDI, where eResearch has focussed on the development of interoperable spatial knowledge systems with dynamic modelling and visualisation capabilities, to assist agricultural research and production, natural resource management, socioeconomic research and municipal planning (e.g. Dahlhaus et al. 2011; 2012; MacLeod et al. 2013; Milne et al. 2014; Thompson et al. 2014).
These systems typically have the following features:
- The data reside with the data managers (ensuring currency and validity)
- They are intuitive to use (similar to Google Maps)
- All forms of data are included (vector, raster, text and multimedia)
- Data downloads are allowed (subject to data custodian's consent)
- Spatial data links to original source (documents and images)
- Spatial data links to real time data (data loggers, webcams)
- They are capable of analysing the interoperable data on the fly
- Interactive 3D visualisations can be created for user-selected scenes
- Users can add, edit or update data (subject to quality assurance and quality control)
- The spatial data and models are credible to the user
The initial flagship project - Visualising Victoria's Groundwater (VVG) - is an online spatial data portal that federates groundwater data for Victoria and is heavily used by consultants, water managers, researchers, students, groundwater users and the global research community. The portal is innovative because it was developed outside of the government and offers real-time access to remote authoritative databases by integrating the interoperable web services they each provide. It includes tools for data querying and 3D visualisations that were designed to meet end-user needs and educate the broader community about a normally invisible resource (Dahlhaus et al. 2012; Dahlhaus et al. 2016b).
The Value Case
The impact of eResearch and digital technologies is only just being measured. In scouring the global literature there are very few published examples of projects that have tracked the usage of the web-portals and the practice changes that has resulted from making data or information available. In Victoria, the impact of the VVG web portal was measured using multidisciplinary research that employed survey instruments, expert reference groups, and internet analytics to explore the extent to which the web portal has supported decision making by the end users (Dahlhaus et al. 2016b). The research found that the portal has changed practices as evidenced by the:
- Primary impact: usage statistics show a steadily growing usage of the site
- Practice impact: surveys confirm that it has changed the way citizens and industry practitioners source their data and the way in which water authorities respond to data requests
- Sector impact: surveys demonstrate that it has changed the way in which the groundwater sector provisions data
While cost savings through efficiency were identified, they have not yet been quantified.CeRDI has embarked on longitudinal research to qualify and quantify the value cases of investment in eResearch. The imperative for initiating this research was partly because it is novel research and partly because the eResearch investors increasingly seek demonstrable justification for their investment.